2. Special Characteristics of ChildrenBECAUSE THEY ARE growing and developing, infants and children are different from adults in composition and metabolism as well as in physiological and biochemical processes. In a period of 26 weeks, or about 6 months, the human concepts grows from microscopic size to recognizable human form weighing almost 500 g (1 pound). At that time, its organs and body systems (cardiovascular, pulmonary, genitourinary, gastrointestinal, neurological, hematological, immunologic, endocrine, and musculoskeletal) are sufficiently mature that extrauterine existence is possible—but survival is very risky. After 3 more months of intrauterine growth (38 weeks of gestation), the average fetal weight increases to 3.5 kg (7.5 pounds), and the organs and body systems become mature enough that adaptation to life outside the uterus is relatively assured. From birth through adolescence, physical growth and functional maturation of the body continue. The rates of physical growth and functional development vary from system to system, organ to organ, and tissue to tissue during this time. Thus, not only do infants and children differ from adults, but at any point during maturation, the individual differs in structure and function from herself or himself at any other age.
This chapter summarizes what is known about these differences. Several aspects of physical growth (structure) and development (functional maturation) are considered in terms of the implications they may have for evaluating the impacts of pesticides on children's health.
Physical development of the body (overall growth), nervous and digestive systems, liver and kidneys, and the proportions of body water and body fat are of special concern in the study of developmental toxicology. Prior to full maturation, damage to an organ or organ system, such as the central nervous system, could permanently prevent normal physical maturation. Also of concern are the physical properties of the toxic substances. For example, water-soluble compounds will be more diluted when the proportion of water in the body is higher, as in infancy, and lipid-soluble substances will be more concentrated in fatty or adipose tissue when the proportion of fat in the body is lower, as may also occur in infancy.
Functional development involves changes in the operational reactions that constitute the process of living, such as the ability to digest and absorb substances in the gastrointestinal tract, to alter their composition in the liver, to develop new metabolic pathways, and to excrete chemicals in the urine. These functions also develop at differing rates, so the organism may respond to chemicals in different ways at different ages. For example, because the filtering function of the kidney develops at a rate different from that of the reabsorptive and secretory functions, the kidney's overall ability to rid the body of toxic substances and the relative ability to excrete substances that are partially reabsorbed or are secreted will vary in a nonlinear fashion with increasing age.
The development of the functions of digestion, absorption, distribution, metabolic alteration, and elimination is of major importance in studies of developmental toxicology. This importance results in part from the possibility that toxicity to a functional system (e.g., glucose homeostasis) prior to its full development may permanently affect that system, resulting in altered function (e.g., glucose metabolism) in the mature animal, and in part from differences in the rates of absorption, metabolism, and excretion and therefore differences in susceptibility to toxicity at different ages prior to maturation.
Behavioral development includes the maturational changes in physical and mental activities associated with the relation of the individual to the environment. Behavioral development has four interrelated aspects: (a) gross motor and fine motor activities; (b) cognitive ability; (c) emotional development; and (d) social development. Alteration in one of these domains can affect the development of each of the other three.
Because of the dependence of behavioral development on physical and functional development, toxic effects occurring before maturation may permanently alter behavioral development. The most commonly encountered and well-known toxicants that can permanently change all four of the components of behavioral development are bilirubin toxicity in the newborn and lead toxicity in the infant or young child. All four aspects of behavioral development are important in studies of developmental toxicology, but much more attention has been given to the first two because they are easier to measure.
The committee's conclusions regarding how infants and children differ from adults with respect to susceptibility to toxicity are based on an extensive literature review. The few available epidemiological studies of chemical toxicity in humans, usually adults, are supplemented by experimental studies in animals. However, much less experimental work has been done on the relationship between body systems and pesticide toxicity in immature organisms than in mature ones. Therefore, data on the relationship between differences in structure and function of the young as they pertain to pesticide toxicity are supplemented with data on the effects of other toxic substances on immature organisms.
Although infant and adult nonhuman animals differ in much the same way that human infants and adults differ, there are substantial interspecies differences among the young. For example, the newborn mouse or rat more nearly resembles the human fetus in the third trimester of gestation than the human infant at birth. On the other hand, the rate of maturation and growth of the mouse or rat after birth is relatively more rapid than that of the human. Thus, cross-species comparisons of potential toxicity for pesticides in the very young animal, although helpful, cannot be used in the same manner that cross-species comparisons are used with adult animals because of differences in developmental patterns. At birth, the dog's brain reaches approximately 8% of its mature weight, the hamster 8%, the rat 15%, the mouse 22%, humans 24%, and the monkey 60% (Himwich, 1973). Subsequent rates of growth are shown in Figure 2-1. Examining the data in another way, as percent of mature weight, human brain weight at 15 to 20 months of age is similar to rat brain weight at 13 to 17 days of age. The rat brain from birth to 26 days is like the hamster brain from 3 to 17 days. For a different variable, the development of γ-aminobutyric acid (GABA) in the cat between the fetal ages of 40 and 44 days is like that of the dog between birth and 10 days of postnatal age (Himwich, 1973).
In some parts of this report, the term development is used to include both functional and behavioral development. Thus when reference is made to "growth and development," the term growth refers to the physical or structural changes associated with the process of maturing, and development refers to the functional and/or behavioral changes that occur during maturation.
GROWTHPhysical growth is a regulated process that represents the sum of the processes of growth of individual cells, tissues, organs, and body systems. These components do not grow at the same rate, but each component has its own rate characteristics. Thus, it is possible to predict the composition of the body from one time to the next; however, the overall composition
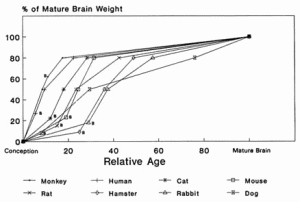
FIGURE 2-1 Differences in growth rate of the brain in several animal species. The relative-age scale depends on the age for mature brain size in each species. At birth, for example, the monkey brain will be 60% of its mature size, the human and mouse brains will be 24 and 22% of their mature size, and the hamster and dog brains will be less than 10% of mature size. Body weight does not reach 50% of its adult value until after 10 years of age, but by about 6 months of age brain weight is half of adult brain weight. Skeletal muscle grows more slowly in mass than total body weight before adolescence but increases in weight more rapidly than does body weight after adolescence. The combined weight of liver, heart, and kidneys increases more slowly than brain weight and is about 50% of that of the brain during early childhood. Liver, heart, and kidney weight reaches 50% of its adult value before adolescence and exceeds brain weight by early adolescence. B, birth. SOURCE: Based on data from Himwich, 1973. Age at brain maturity: monkey, 4 yrs.; human, 12.5 yrs.; mouse, 90 days; rat, 120 days; hamster, 60 days; dog, 120 wks.
is never the same from moment to moment until growth processes are complete.
Normal Human GrowthAfter birth of the full-term human infant, growth occurs at an average rate of 800 g/month or at an incremental rate of 25% of total body weight per month. Because this rate soon slows, the actual doubling of birth weight takes 5 to 6 months. At 1 year, the infant reaches almost three times its birth weight (Table 2-1). After the first year, growth proceeds at about 200 g/month. A peak gain is reached at adolescence: 500 to 600 g/month for boys and somewhat less for girls (Cheek, 1968a).
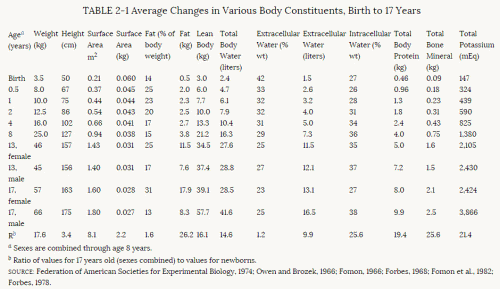
TABLE 2-1 Average Changes in Various Body Constituents, Birth to 17 Years
Growth in body proportions also changes dramatically during childhood as it does in utero. By 2 years of age, at four times birth weight, the toddler achieves about 20% of adult weight and about 50% of adult height; skull circumference and brain size will already be near their adult values. At birth, the length of the head is 25% of body length; by adulthood the head is only about 14% of the length of the body. Thus, growth proceeds from the head downward. The midpoint of the newborn's body is at midabdomen, but by adulthood it is at the junction of the legs with the trunk.
During infancy and adolescence, children are growing and adding new tissue more rapidly than during any other period in their postuterine life, but their various organs, tissues, and metabolic processes are maturing at different rates. For example, the neuronal cell population is relatively complete by 2 years of age, but full myelination of neuronal tissue is not complete until their 18th year. The brain achieves 50% of its adult weight by 6 months of age, whereas approximately 50% of adult stature is not reached until 2 years of age. In contrast, 50% of the adult weight of the liver, heart, and kidneys is not reached until the children are about 9 years old, and the same point in growth of skeletal muscle and total body weight is not attained until approximately the 11th year of age. Thus, as the child grows, his or her body consists of differing proportions of various tissues and organs that comprise the body. Various tissues—brain, skeletal muscle, liver, heart, and kidney—have different metabolic rates and biochemical pathways, and their changing physical proportions will alter the disposition of xenobiotic compounds over time.
The major periods of rapid growth include infancy and puberty. Growth is most rapid in the combined period of in utero development plus infancy and puberty. From birth to 4 years of age, the rate of growth decreases from 50 cm/year to the childhood value of about 10 cm/year. During puberty, the rate of linear growth increases to approximately 12 cm/year. These periods of rapid growth are believed to be susceptible to adverse influence of toxicants (Karlberg, 1989).
The hypothalamus, pituitary glands, gonads, and other tissues are involved in the control and expression of growth during puberty. Growth hormone (GH), both directly and through stimulation of the somatomedins, plays a major role in growth through endocrine, autocrine, and paracrine mechanisms. GH deficiency is associated with impaired growth, and GH excess with increased growth. The androgens and estrogens, through anabolic and other endocrine mechanisms, also modulate growth at puberty. High levels of estrogens (or estrogen-like molecules such as some pesticides) can, through effects on the growing bone, decrease the optimum height attained in adulthood or rate of linear growth during puberty.
Human Compared to Animal InfantsThe newborn rabbit, rat, mouse, and hamster can double their birth weights in less than 1 week, much faster than the human infant can (Altman and Dittmer, 1962). These different growth velocities may alter the toxicity of pesticides and other chemicals among different species of infant animals. In various theories of carcinogenesis, it has been postulated that the rapidity of DNA synthesis and cell proliferation affects on carcinogenicity or other toxic manifestations of chemicals. Therefore one might expect the biological effect (either positive or negative) to be more pronounced in the more rapidly growing animal—e.g., the dog, rabbit, or rat—than in the human infant. The effects on the mouse or hamster should be less pronounced than those on the dog, rabbit, or rat but still more pronounced than those on the human infant. However, if toxicity is related to absolute rates of growth, the order of increased sensitivity would be the hamster (36 days for a 10-fold increase in birth weight), the mouse (54 days), the rabbit (80 days), the rat (160 days), the dog (165 days), the monkey (1,000 days), and humans (5,000 days). This issue is complex, but in the absence of other factors, direct carcinogens are more potent in rapidly growing animals (Cohen and Ellwein, 1991; Weinstein, 1991).
Toxicologic Implications of Growth in Cell Numbers and SizeThe rapid growth from conception through infancy in both animals and humans is achieved primarily by an absolute increase in the number of cells in the body (hyperplasia). After infancy, the bulk of growth is the result of increase in cell size (hypertrophy). The major exception is in the growth related to increased secretion of a series of hormones in adolescence. The change from hyperplasia to hypertrophy has major implications for adult form and size. Because of the possibility of a permanent reduction in total cell number, factors that alter growth prior to 2 years of age, even if only transient, are much more likely to result in diminished adult size than similar transient effects that occur after 2 years of age. An example of this phenomenon is the permanent reduction in body size that results from congenital rubella.
During the period of hyperplasia, which varies in duration from one organ to another, the increased rate of DNA/RNA replication and of protein synthesis may have different implications for toxicity. In a study of the age-related sensitivity of 3-, 7-, 11-, and 29-week-old rats to the induction of anemia, phenylhydrazine (PHZ) produced more marked anemia in the older animals on a milligram-per-kilogram basis (Vesell, 1982). The author postulated that the ''resistance" of the younger animals could have been secondary to their increased rate of red blood cell proliferation. When a dosage based on surface area was used, the results continued to demonstrate a proportionate age-related sensitivity to PHZ.
Increased rates of cell proliferation can be associated with negative as well as positive outcomes. In the mammary gland, the proliferation of cells is greatest at the time of menarche—the first menstrual period—and the greatest risk for carcinogenesis from radiation is when the radiation occurs immediately before and after that event (Norman, 1982; Miller et al., 1989). On the other hand, radiation during infancy for treatment of an enlarged thymus also increased the risk of breast cancer 30 to 40 years later (Hildreth et al., 1989). The role of cell proliferation in carcinogenesis remains unclear and may depend on a variety of other factors (Moolgavkar and Venzon, 1979; Moolgavkar and Knudson, 1981; Finhorn, 1982; Rajewsky, 1985; Moolgavkar, 1988; Moolgavkar et al., 1988, 1990.a,b; Cohen and Ellwein, 1990, 1991; Moolgavkar and Luebeck, 1990; Cohen et al., 1991).
In one study, 3- and 14-month-old rats were given intravenous doses of N-nitrosomethylurea (NMU), a known carcinogen (Anisimov, 1981). The 3-month-old rats received doses of 200 or 100 mg/kg of body weight (bw) (30 or 15 mg per rat) (groups 1 and 2, respectively), and the 14-month-old animals received 100 mg/kg bw (30 mg per rat) (group 3). The animals were observed throughout their life span, and tumor incidence was compared with that in a nontreated control group (group 4). Mammary adenocarcinomas occurred only in the rats treated at 3 months of age: 71% of group 1 and 32% of group 2 animals. Cervicovaginal sarcomas and carcinomas were found only in the group 3 animals injected at 14 months of age. Kidney tumors occurred in groups 1, 2, and 3 but were most common (54%) in the group 2 animals (the 3-month-old rats receiving the lower dose of NMU). The incidence of kidney tumors in group 1 was 10% and in group 3, 17%; there were none in the control group. Neoplasia of the hematopoietic system was found equally in groups 1, 2, and 3 (33%, 32%, and 25%, respectively, compared with 9% in the controls). The author postulated that the younger rats developed mammary and renal tumors more readily than the older animals because the proliferation of mammary gland cells and renal epithelium had decreased with age. The lack of mammary tumors in the animals treated at 14 months may reflect the insufficient number of remaining months of life to allow for development of the tumors. However, the growth of mammary tissue generally precedes the growth of vaginal/uterine tissue in most species. The older animals developed more uterine, cervical, and vaginal tumors. The higher level of estrogens at their age (14 months) was believed to be associated with more rapid proliferative activity of the target tissues (Anisimov, 1981).
In a study by Drew et al. (1983) of the effect of age and duration of exposure on the carcinogenicity of vinyl chloride (VC) in female rats, hamsters, and mice, younger animals were generally more affected than older ones. Beginning at about 2 months of age, animals were exposed to VC for 6, 12, 18, and 24 months. Other animals were exposed for 6 or 12 months beginning 6, 12, or 18 months later than the original groups. For all animals, the life span was shortened when exposure began at 2 months of age, regardless of duration of exposure (except for rats exposed for 6 months). Exposures beginning 6, 12, or 18 months later did not shorten the life span except in B6C3F1 mice, which survived about 315 days from the onset of exposure regardless of the age at which exposure was initiated.
The incidence of tumors was also directly related to age of onset of exposure and duration of exposure, but age of onset appeared to be the dominant factor. One exception to this finding was that hepatocellular carcinomas were more prevalent in rats whose 6-month exposure began 6 months later than that of the group treated at 8 weeks. The incidences of hemangiosarcomas and mammary gland adenocarcinomas, as well as neoplastic nodules, were all related to the earlier age of exposure. The greatest incidence of hemangiosarcomas and stomach adenomas was observed in hamsters whose 6-month exposures began at the earliest ages. Longer exposures did not change the incidence. Similar findings were noted in the mice. However, the incidence of hemangiosarcomas was not affected by age in the B6C3F1 mice, but was related to age in the Swiss mice.
In general, exposures beyond 12 months did not change the incidence of tumors in rats, and 6-month exposures of hamsters and mice were sufficient to achieve maximum tumor incidence with only two exceptions. Of note is the finding that the incidence of hemangiosarcomas and stomach adenomas in hamsters was reduced by exposures greater than 6 months. However, Drew et al. (1983) postulated that the shortened life span resulting from the longer exposures could have caused the animals whose exposure began at 2 months to die before their tumors could be expressed. The authors pointed out that animals exposed early in life must be allowed to live out their full life span if appropriate tumor incidence is to be ascertained. When this was done, it was quite apparent that the animals exposed at the youngest age had, with an occasional exception, the highest incidence of a variety of tumors. These studies lend support to the concept that the rate of cell proliferation (greatest in the youngest animals) is a contributing factor to the carcinogenic effect of VC. Similar conclusions were drawn by Swenberg et al. (1992).
Toxicologic Implications of the Growth and Development of OrgansThe pattern of the rate growth of the various organs in the human infant varies from the pattern of the overall body growth rate. Figure 2-2A illustrates the growth rate for several organs in humans. The thymus grows most rapidly initially, and throughout most of childhood exceeds its adult size. The brain approaches adult size early in childhood, although behavioral development continues for many years. The uterus and testes grow slowly until adolescence, and the growth of the ovaries is similar to that of the kidney and spleen and to increase in total body weight. Figures 2-2B and 2-2C present growth curves for some of the same organs in the rat and mouse. Each of these species has a different pattern of specific organ growth, and these differences complicate comparative studies between species. Much less is known about the rate of functional development of various organs. The age period in which specific organs or tissues undergo their most rapid rate of development and the age at which development is completed have major implications for studies of toxicity to those organs in growing animals. Toxicity that is dependent on rates of cell proliferation (DNA/RNA replication and protein synthesis) might affect different tissues or organs at various stages of animal growth. Furthermore, whether the effect generated permanently alters the pattern of adult function, depresses adult function, or has no permanent effect may depend on whether the particular organ or tissue is still evolving or has reached its adult capacity at the time of exposure. Knowledge of the developmental pattern for various tissues and organs in animals used for studying pesticide toxicity is important in determining potential target sites and toxic end points in humans.
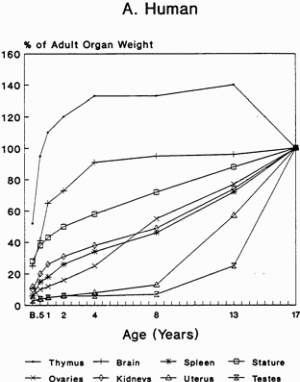
FIGURE 2-2 Organ development and stature or body weight as percentage of adult values by age in the human (A), rat (B), and mouse (C).
SOURCE: Based on data from Altman and Dittmer, 1962.
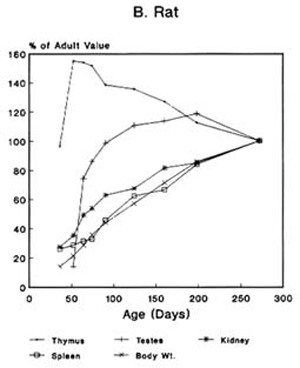
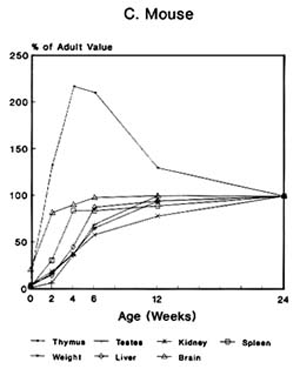
On another level, cellular functions within the body and within organs change with the period of overall growth. From early gestation to early infancy, blood cells are formed in the liver and spleen, but by late infancy only the bone marrow functions in the initial development of blood cells. However, "the volume of bone marrow in newborns is in fact so large that it is nearly equal to the marrow space occupied by hematopoietic cells in adults" (Nathan, 1989). For compounds that are potentially toxic to the hematopoietic system, the anatomic location and the relative size of the system may determine the degree of toxicity. Substances that accumulate in the liver or spleen in the young infant could have a direct effect on hematopoiesis—an effect that might be absent in the older child or adult. Similarly, the relatively large volume of hematopoietic tissue in the young could contribute to a more profound effect for substances that are widely distributed in the body but could reduce toxicity through dilution of compounds that concentrate in such tissue.
In several portions of the brain, the nerve cells (or neurons) that will ultimately be near the surface of the brain (in the cortex) must migrate from more central locations to the cortex during late fetal and early infant life (Rakic, 1970, 1972). This is only one of many developmental processes taking place in the brain that could be disrupted by substances toxic to the central nervous system (Langman et al., 1972). Although the developmental sequences of tissue organization and cellular maturation are similar in various animal species, the specific rate of each sequence relative to other sequences and across species differs significantly (Rodier, 1980). The myelination of nerve tracts in the spinal cord and peripheral nerves is a process that continues throughout childhood. Incomplete myelination of nerve fibers could alter their response to xenobiotic agents. The cumulative dose of cisplatin "that causes peripheral neuropathy tends to be higher in children and younger patients than in the elderly" (Legha, 1990). Whether the decreased sensitivity in the child is related to incomplete myelination or to other metabolic differences is unknown. Thus, the impact of toxic products can produce quite different outcomes that vary both with time and with species.
Toxicologic Implications of Changes in Body CompositionThe human infant is compositionally immature at birth. The infant's body is unlike the adult's in terms of body water and the relation of skeletal mass to other lean body tissue. Table 2-1 provides values for various body constituents at ages that represent significant developmental periods in the life of a child. The bottom row presents the value ratios for 17 year olds compared with newborns. From birth to age 17, body weight increases about 18-fold, stature slightly more than 3-fold, and surface area about 8-fold. Total body fat increases about 36-fold in the female and about 17-fold in the male. Body water increases about 15-fold, but extracellular water only 10-fold. Total body protein increases almost 20-fold, whereas bone mineral increases by 25-fold and total body potassium by about the same amount as total body protein because protein is located primarily in cells and potassium is primarily an intracellular ion.
The rate at which various body constituents increase and their relative proportions in the body shift during childhood (see Table 2-1). For example, total body fat accumulates most rapidly in infancy and again in adolescence, especially in the female. Bone mineral also increases markedly in adolescence—especially during the male's major growth spurt in late adolescence. Another relationship that has been known for many years is the correspondence between the extracellular fluid volume and the surface area of the body. Both of these sets of values also have a linear relationship to metabolic rate for most of childhood.
The implications of these changes in body composition for pesticide toxicity are not well defined experimentally, but one can hypothesize a number of possibilities. The relatively larger extracellular fluid volume of the infant would result in a somewhat greater dilution if the infant were to ingest equivalent amounts (on the basis of body weight) of water soluble substances. Alternatively, lipid-soluble substances given in equivalent amounts on a body weight basis would be more concentrated in the fat of the young child because of the lower amount of fat per kilogram of body weight. Similarly, compounds that have an impact on bone growth would be more concentrated per unit of bone mass because of the bone's relatively smaller size per unit of body weight in the infant and child.
Premature and full-term infants differ from older children and adults in their body composition. Whereas the premature infant may be 85% water, the lean body mass of the full-term infant is 82% water, compared with 72% water in the lean (fat-free or non-fat-containing) body mass of the adult. Most of the "excess" water in the infant is extracellular (Forbes, 1968). Thus, the overall amount of organ tissue per unit of whole body mass is less in the infant. One exception is the liver, which in the child is relatively larger per unit of body weight than in the adult. Several investigators have postulated that this relatively larger size of the liver could play a role in the capacity of the young child to metabolize drugs such as phenylbutazone and antipyrine more rapidly than the adult (Coppoletta and Wolbach, 1933; Alvares et al., 1975; Vesell, 1982). In addition, the organs themselves contain more water than do the organs of adults (Dickerson and Widdowson, 1960; Widdowson and Dickerson, 1960, 1964; Dickerson, 1962). Brain water decreases from 90% in the infant to 77% in the adult (Altman and Dittmer, 1973). Liver water decreases from 78% to 71% and kidney water from 84% to 81% (Widdowson, 1968). Although the total water content of skeletal muscle is relatively unchanged, the extracellular water of muscle decreases from 35% to 18%, but the intracellular water increases from 45% to 61%. The change in intracellular water is to a large extent related to an increase in cell size.
The changes in body water compartment sizes with increasing age may bear some relationship to changes in pesticide toxicity, depending on the distribution of the toxic compound. Those agents that are water soluble and distributed extracellularly will be more diluted in the youngest animals, or in human infants and small children, for comparable exposures on a milligram-per-kilogram basis. Thus one might expect toxicity to vary with age for water-soluble compounds. If the compound or its toxic metabolite is distributed intracellularly, the relatively decreased volume of intracellular water in the young would lead to toxicity varying indirectly with age. These age-related differences in toxicity emphasize the importance of ascertaining the distribution of water-soluble toxic materials within the specific tissue water compartments, extracellular or intracellular.
Cell size in infancy is relatively small. Thus, there is proportionately more cell membrane per unit of cell mass in the infant than in the adult (Cheek, 1968b). The effect of the relatively greater cell membrane and, therefore, of cell surface area compared to cell mass in the infant and young child has not been explored, but one might postulate that these proportions could increase the sensitivity of the cells of the young to compounds that act primarily at the level of the cell membrane.
DEVELOPMENTDevelopment, as used in this context, refers to the functional maturation of various cells, tissues, organs, organ systems, and the organism as a whole. As noted previously, the changes in function follow patterns similar to but not identical with physical growth. Functional development, like physical growth, is established to a large extent by genetic mechanisms. However, alterations in the patterns of functional development may be more readily modified by external (environmental) factors than the patterns of physical growth. In addition, there may well be external factors that modify growth but do not affect development and vice versa.
Genetics, Development, and the EnvironmentUnderstanding infant and child development in relation to the toxicity of pesticides requires an understanding of the constantly evolving interaction between a person's genetic endowment, developmental processes, and the environment. The infant or small child's phenotype (characteristics produced through interaction between genetic properties and environment) more closely resembles its genotype (genetic properties) than is true for the adult. At conception, each individual is genetically unique. From the time of conception, environmental factors may alter the genotype to produce a different phenotype. As the phenotype changes, it may alter the environment and be further altered by the environment. Specific enzyme systems may be enhanced, delayed, or altered permanently in their development by environmental factors. In addition, environmental exposures may impair, alter, or delay the development of some biochemical or physiological systems. Thus, not only will the programmed development of enzyme function alter the responses to xenobiotic compounds in immature, compared with mature, organisms, but environmental exposure to such substances before maturity may further modify response at a later time, or in some cases, throughout the life span. The specific stage in tissue or organ development when environmental factors can modify the cells to produce an effect apparent only in later life are termed critical periods of development. For example, giving insulin or glucosamine to newborn animals may permanently alter the mature animal's insulin levels and blood glucose values (Csaba and Dobozy, 1977; Csaba et al., 1979). Another example is the elevation of serum bilirubin in the human neonate, which produces altered brain function that becomes evident as the child matures. Similar elevations of serum bilirubin after infancy fail to produce these changes. Some of the damage to the central nervous system resulting from exposure to low levels of lead may not be apparent until the development of more mature functions in test animals (Csaba et al., 1979) or of such skills as reading and arithmetic in children. Similarly, neonatal exposure to diethylstilbestrol may produce effects later in life in the reproductive system (adenocarcinoma of the vagina and impaired function of the reproductive and immune systems) (Kalland, 1982).
As a general rule, compounds that interact with some genetic component of an individual are likely to be more active and cause greater impact on the young, whereas those compounds that depend for their activity on the development of acquired characteristics—such as elevated blood pressure, atherosclerosis, and loss of renal function—will be more active in the elderly. For example, the red blood cells of older mice are more easily damaged by oxidants, possibly because of acquired changes in their cell membrane (Tyan, 1982) and impaired ability to withstand oxidant stress. Similarly, the increasing presence of chromosome aberrations in the cells of the older person, perhaps because of longer exposure to toxic substances in the environment, may contribute to the increased carcinogenic susceptibility of the elderly (Singh et al., 1986).
MetabolismThe metabolic rate, a measure of the total energy expenditure of an organism, increases as the size of the organism increases. However, when energy expenditure or energy intake is evaluated per unit of mass, it decreases with increasing body size. Thus, the metabolism of exogenous substances administered in terms of body size will generally be more rapid in the immature than in the mature animal. This may have important implications for toxicity of pesticides—decreasing toxicity when the parent compound is the toxic agent and increasing toxicity when a breakdown product is the toxic substance. However, there is a variety of other aspects of changes in metabolism that can also act to modify toxicity.
The infant's body surface area per unit of body mass is two to three times greater than the adult's. This relatively large surface area per unit of mass is associated with a metabolic rate that is more than twice the adult's on a weight basis but closer to the adult value when compared per unit of surface area. Because food and water intake is related to metabolic rate, the infant's consumption will be greater than the adult's on a weight basis, but total energy consumption will be more nearly comparable to the adult's if surface area is the basis of comparison. Thus, infants differ from adults, not only in specific metabolic and pharmacokinetic measures, but also in overall metabolic rate.
Because of differences in the types of food consumed by the young (see Chapter 5), the amount of a food consumed, with its related additives, preservatives, and contaminants, may be 1 order of magnitude higher or lower for children than for adults per unit of body weight. The percent absorption varies with the development of the gastrointestinal system. The volume of distribution also depends on the degree of maturity as well as on the solubility characteristics of the substance. Because of developmental changes in enzyme activity, rates of deactivation or activation are related to the stages of maturation. The number of cell membrane receptors and the degree of protein binding are similarly variable with age. Rates of excretion by the liver and kidneys depend on both the overall organ development and the differential rates of maturation of specific organ components. For example, the development of the filtration function of the kidney is slower than the development of the reabsorption and secretory functions. Finally, the cellular response itself is dependent on intracellular maturation and differentiation. Thus, the human infant or infant animal may respond quite differently from the adult of its species to many xenobiotic substances. There is no reason to believe that these differences in response would not be equally applicable to pesticides.
Developmental Toxicity StudiesBecause so many bodily functions are at various stages of development throughout infancy and early childhood, toxic effects of chemical agents during these age periods not only produce the same sorts of direct injuries to established organ tissues and functions seen in adults, but also have the potential to affect the later development of anatomic, physiologic, and metabolic processes.
During organogenesis, functional integrity does not necessarily coincide with morphological maturity, and relative organ size varies as development proceeds. Because of the evolving development of various organs and tissues, the effect of exposure to toxic substances will vary in a complex way with the age at exposure. Substances that are toxic to adults may have minimal effects at one stage of development, but at another stage these same substances may produce permanent damage to the organism or may be lethal. Such effects are particularly prominent for the central nervous system. For example, radiation treatment for medulloblastoma resulted in major cognitive problems at a later age for children less than 4 years old at the time of radiation, minimal problems later if treatment occurred at 5 to 7 years of age, and no residual cognitive difficulties when radiation was administered at 8 years and older (Chin and Maruyama, 1984).
The infant kidney is immature at birth, and the relatively poor glomerular filtration rate leads to delayed drug excretion and, therefore, an increased likelihood of toxicity (Kleinman, 1982). In the liver, the capacity to detoxify drugs by the process of conjugation develops slowly and is a major factor contributing to the toxicity of chloramphenicol in infants (Vesell, 1982).
The complexity of age-related effects of exposure for a specific tissue was demonstrated by Fleisch (1980), who identified the following factors that could change with age and thus alter the age-related sensitivity of blood vessels to various drugs:
FIGURE 2-3 Changes in the arterial smooth muscle ß-adrenergic system with age. The overall decrease in arterial smooth muscle relaxation with isoproterenol stimulation as the organism ages can be related to three separate changes in the model. SOURCE: Based on data from Fleisch, 1980.
• the number of impinging nerve cells,
• the number of drug receptors per cell,
• the relative proportion of one type of receptor to others,
• the cell membrane composition,
• the second messenger systems, and
• the concentration of reactive proteins.
The complexity of the age-related changes in vascular responsiveness is illustrated by analysis of the changes in contractile function of the arterial smooth muscle in the rat. The responsiveness of the venous smooth muscle to the stimulant isoproterenol does not change with age, whereas the responsiveness of the arterial smooth muscle increases in the postnatal period and then declines with increasing maturity. In the simplified model used to exemplify the response, isoproterenol combines with its receptor in the cell membrane and then stimulates a chemical messenger such as cyclic adenosine monophosphate (AMP), which in turn reduces the contractility of the structural protein (see Figure 2-3). In this model the affinity of isoproterenol for the receptor does not change with age, but the number of receptors per cell does decrease with age. The sensitivity of the chemical messenger to receptor stimulation does not change with maturity, but the sensitivity of the contractile protein decreases in the older rats. In addition, the overall contractile property of the structural protein shows a decline with maturation. Incidentally, there are no comparable unambiguous data on the changes in alternative stimulants with age, although age-related changes in function have been demonstrated.
Without detailed knowledge of all such age-related physiological changes and their potential interactions, it is impossible to extrapolate the impact of xenobiotic effects from mature to young animals. It is therefore necessary to examine such effects in immature animals of various ages.
A further complication is that developmental aspects of organs, tissues, and cells in the young may increase sensitivity to some drugs and decrease sensitivity to others. In fact, for the same compound one aspect of the immature organism may increase sensitivity and another aspect may decrease sensitivity. For example, the immaturity of some metabolic functions may lead toprolongation of the half-life of a toxic substance, but the larger volume of extracellular water may reduce its concentration. Reduced numbers of binding sites on target cells could also reduce the toxicity of the substance.
After reviewing a variety of studies of developmental toxicity, Calabrese (1986) concluded that there is no systematic way to predict differences in toxicity related to age. Because of differences in mechanisms of toxicity, rates of metabolism, enzyme development, stages of organ system development (some of which are more resistant to damage than others), and relative proportions of organ mass, it is unlikely that a predictive model of toxicity and development will evolve in the near future (Calabrese, 1986).
In 1983, Anisimov examined the age of animals as it relates to their sensitivity to carcinogens. He discussed four factors that may alter sensitivity on the basis of age:
• changing activity of carcinogen-metabolizing enzymes,
• changes in binding affinity,
• age-related alteration in the accuracy of DNA repair, and
• variation in proliferative activity of target tissues as a function of age and of secondary modifiers of proliferation such as hormones and tissue-specific growth factor.
Because epidemiological data provide contradictory relationships between age at exposure and the development of cancer, and because such relationships vary with specific tumors, Anisimov examined 52 studies of chemical-induced carcinogenesis in animals of different age groups. In a tabulation of these studies for the effect of aging on latency, incidence, and tumor size, he noted that aging increased chemical carcinogenesis in 28 studies, decreased it in 19 studies, and had no effect in 5 studies. Analysis by animal species, target tissue, or chemical agent did not reveal any specific pattern (Anisimov, 1983). In his own studies of N-nitrosomethylurea in rats of various ages, Anisimov (1983) made several observations associated with increasing age at exposure: a decrease in tissue sensitivity to the action of the carcinogen in the mammary gland and kidney; an increased sensitivity in the cervix, uterus, and vagina; and no influence in the hematopoietic system (Anisimov, 1981).
If one were to examine the entire life span at many points, however, it is likely that sensitivity to pesticides and other toxic agents would not follow a regular progression in toxicity from infancy to senescence. One example is a study of the toxicity of 14 pesticides in mallard ducks at 1.5, 7, 30, and 180 days of age. In no case was there a regular progression of toxicity relative to age (Hudson et al., 1972). In all cases but two, there was either an initial decrease in toxicity followed by an increase (four cases) or an initial increase in toxicity in the earliest ages followed by a decrease (eight cases).
CONCLUSIONS AND RECOMMENDATIONSInfants and children from the beginning of the third trimester of pregnancy until 18 years of age are the subject of this report. This age span was selected to cover the period of development from the onset of possible extrauterine existence to the age of maturation of essentially all biological systems. Reproductive toxicology and teratology are not a focus of this study.
The chapter discusses maturation in terms of physical growth, physiological and behavioral development, and the impact of the changing patterns of anatomical structure and function on the toxicity of chemicals in the immature individual. The changes do not begin at similar times or proceed at similar rates. At present, toxicity data on mature animals are insufficient for extrapolation to immature animals.
Conclusions• Human infants and children differ from human adults not only in size but also, and more importantly, in the relative immaturity of biochemical and physiological functions in major body systems; body composition in terms of proportions of water, fat, protein, and mineral mass, as well as the chemical constituents of these body components; the anatomic structure of organs; and the relative proportions of muscle, bone, solid organs, and brain. These structural and functional differences between neonates and adults can potentially influence the toxicity of pesticides, due to qualitative and quantitative alterations in the magnitude of systemic absorption, distribution, binding, metabolism, interaction of the chemical with cellular components of target organs, and excretion. Without detailed knowledge of all age-related physiological changes and their potential interactions, it is not possible to extrapolate the impact of xenobiotic effects from mature to young animals.
• Whatever the target tissue or organ may be, some responses to pesticides may alter physiological processes that influence the uptake and metabolism of food constituents needed for optimal growth and development. Interference with such processes could result in disorders of overall growth or functional disorders of specific organ systems. It is often not known which developmental stage of individual biochemical systems, tissues, or organs will enhance, diminish, or not alter the infant's or child's sensitivity to the toxic effects of specific pesticides. In the experimental setting, therefore, the stage of growth and development of laboratory animals is a critical variable in evaluating the toxicity of pesticides.
• There are, however, specific periods in development when toxicity can permanently alter the function of a system at maturity. These special windows of vulnerability (critical periods) are often found in the early months of human pregnancies; however, some systems (e.g., the central nervous, immunologic, reproductive, and endocrine systems) continue to mature and may demonstrate particular sensitivity during the postnatal period.
• If a compound's toxicity is age related in one species, it is reasonable to assume that there will be an age relationship in other species in which the compound is toxic.
Recommendations• Care must be taken when selecting an appropriate animal model for investigating the toxic effects of pesticides in infants and children, interpreting the data, and extrapolating the data from young animals to young humans. Toxicity in young rodents can vary substantially over a period of days, since maturation occurs so rapidly in these animals.
• In the evaluation of pesticide toxicity for immature animals, overall growth should be evaluated by measurement of growth rates and adult size at maturity.
• Studies should be conducted to examine age-related physiological changes and their potential interactions in immature animals of various ages.
• Because of the variable rates of organ development within and between species, specific organ system development should be evaluated by functional measures specific to each organ system. For example, if a chemical interfered with glomerular development in the kidney to the extent that the glomerular filtration rate were reduced by 50%, such an effect would not be likely to become apparent until maturity, and only then would the effect be documented by actual measurement of that specific function in the mature animal.
REFERENCESAltman, P.L., and D.S. Dittmer, eds. 1962. Growth—Including Reproduction and Morphological Development. Washington, D.C.: Federation of American Societies for Experimental Biology.
Altman, P.L., and D.S. Dittmer, eds. 1973. Chemical composition of nervous tissue. Pp. 1206–1230 in Biology Data Book, 2nd edition, Vol. 2. Bethesda, Md.: Federation of American Societies for Experimental Biology.
Alvares, A.P., S. Kapelner, S. Sassa, and A. Kappas. 1975. Drug metabolism in normal children, lead-poisoned children, and normal adults. Clin. Pharmacol. Ther. 17:179–183.
Anisimov, V.N. 1981. Modifying effects of aging on N-methyl-N-nitrosourea-induced carcinogenesis in female rats. Exp. Pathol. 19:81–90.
Anisimov, V.N. 1983. Role of age in host sensitivity to carcinogens. International Agency for Research on Cancer. Sci. Publ. 5:99–112.
Calabrese, E.J. 1986. Age and Susceptibility to Toxic Substances. New York, Chichester, Brisbane, Toronto, Singapore: Wiley-Interscience Publication, John Wiley and Sons, Inc.
Cheek, D.B., ed. 1968a. A new look at growth. Pp. 3–18 in Human Growth: Body Composition, Cell Growth, Energy and Intelligence. Philadelphia: Lea and Febiger.
Cheek, D.B., ed. 1968b. Muscle cell growth in normal children. Pp. 337–351 in Human Growth: Body Composition, Cell Growth, Energy and Intelligence. Philadelphia: Lea and Febiger.
Chin, H.W., and Y. Maruyama. 1984. Age at treatment and long-term performance results in medulloblastoma. Cancer 53:1952–1958.
Cohen, S.M., and L.B. Ellwein, 1990. Proliferative and genotoxic cellular effects in 2-acetylaminofluorene bladder and liver carcinogenesis: Biological modeling of the ED01 study. Toxicol. Appl. Pharmacol. 104:79–93.
Cohen, S.M., and L.B. Ellwein. 1991. Genetic errors, cell proliferation, and carcinogenesis. Cancer Res. 51:6493–6505.
Cohen, S.M., D.T. Purtilo, and L.B. Ellwein. 1991. Pivotal role of increased cell proliferation in human carcinogenesis. Mod. Pathol. 4:371–382.
Coppoletta, J.M., and S.B. Wolbach. 1933. Body length and organ weights of infants and children. Am. J. Pathol. 9:55–59.
Csaba, G., and O. Dobozy. 1977. The sensitivity of sugar receptors: Analysis in adult animals of influences exerted at neonatal age. Endokrinologie 69:227–232.
Csaba, G., O. Dobozy, G. Lazary, and G. Kaizer. 1979. Possibility of long-lasting amplification of insulin receptors by a single treatment of newborn age. Acta Physiol. Acad. Sci. Hung. 53:487–492.
Dickerson, J.W.T. 1962. Changes in the composition of the human femur during growth. Biochem. J. 82:56–61.
Dickerson, J.W.T., and E.M. Widdowson. 1960. Chemical changes in skeletal muscle during development. Biochem. J. 74:247–257.
Drew, R.T., G.A. Boorman, J.K. Haseman, E.E. McConnell, W.M. Busey, and J.A. Moore. 1983. The effect of age and exposure duration on cancer induction by a known carcinogen in rats, mice and hamsters. Toxicol. Appl. Pharmacol. 68:120–130.
Federation of American Societies for Experimental Biology. 1974. Blood and other body fluids. Pp. 1751–2041 in Biological Handbooks: Biology Data Book, Second Edition. Bethesda, Md.: Federation of American Societies for Experimental Biology.
Finhorn, L. 1982. On codevelopmental biology and medicine. J. Int. Soc. On codevel. Biol. Med. 4:219–229.
Fleisch, J.H. 1980. Age-related changes in the sensitivity of blood vessels to drugs. Pharmacol. Ther. 8:477–487.
Fomon, S.J. 1966. Body composition of the infant. Part I: The male reference infant. Pp. 239–246 in Human Development, F. Falkner, ed. Philadelphia: W.B. Saunders.
Fomon, S.J., F. Brachka, E.E. Ziegler, and S.E. Nelson. 1982. Body composition of reference children from birth to age 10 years. Am. J. Clin. Nutr. 35:1169–1175.
Forbes, G.B. 1968. Changes in body water and electrolyte during growth and development. Pp. 80–86 in Body Composition in Animals and Man, Proceedings of a Symposium held May 4, 5, and 6, 1987 at the University of Missouri, Columbia, Publication 1958. Washington, D.C.: National Academy of Sciences.
Forbes, G.B. 1978. Body composition in adolescence. Pp. 239–272 in Human Growth, Vol. 2: Postnatal Growth, F. Falkner and J.M. Tanner, eds. New York: Plenum.
Hildreth, N.G., R.E. Shore, and P.M. Dvoretsky. 1989. Risk of breast cancer after irradiation of the thymus in infancy. New Engl. J. Med. 321:1281–1284.
Himwich, W.A. 1973. Problems in interpreting neurochemical changes occurring in developing and aging animals. Prog. Brain Res. 40:13–23.
Hudson, R.H., R.K. Tucker, and M.A. Haegele. 1972. Effect of age on sensitivity: Acute oral toxicity of 14 pesticides to mallard ducks of several ages. Toxicol. Appl. Pharmacol. 22:556–561.
Kalland, T. 1982. Long-term effects on the immune system of an early life exposure to diethylstilbestrol. Pp. 271–241 in Banbury Report 11: Environmental Factors in Human Growth and Development, V.R. Hunt, M.K. Smith, and D. Worth, eds. New York: Cold Spring Harbor Laboratory.
Karlberg, J. 1989. On the construction of the infancy-childhood-puberty growth standard. Acta Paediatr. Scand. Suppl. 356:26–37.
Kleinman, L.I. 1982. The effect of lead on the maturing kidney. Pp. 153–171 in Banbury Report 11: Environmental Factors in Human Growth and Development, V.R. Hunt, M.K. Smith, and D. Worth, eds. New York: Cold Spring Harbor Laboratory.
Langman, J., M. Shimada, and P. Rodier. 1972. Floxuridine and its influence on postnatal cerebellar development. Pediatr. Res. 6:758–764.
Legha, S.S. 1990. Letter to the editor. New Engl. J. Med. 323:65.
Miller, A.B., G.R. Howe, G.J. Sherman, J.P. Lindsay, M.J. Yaffe, P.J. Dinner, H.A. Risch, and D.L. Preston. 1989. Mortality from breast cancer after irradiation during fluoroscopic examinations in patients being treated for tuberculosis. New Engl. J. Med. 321:1285–1289.
Moolgavkar, S.H. 1988. Biologically motivated two-stage model for cancer risk assessment. Toxicol. Lett. 43(1–3):139–150.
Moolgavkar, S.H., and D.J. Venzon. 1979. Two-event models for carcinogenesis: Incidence curves for childhood and adult tumors. Math. Biosci. 47:55–77.
Moolgavkar, S.H., and A.G. Knudson. 1981. Mutation and cancer: A model for human carcinogenesis. J. Natl. Cancer Inst. 66(6):1037–1052.
Moolgavkar, S.H., and G. Luebeck. 1990. Two-event model for carcinogenesis: Biological, mathematical, and statistical considerations. Risk Anal. 10:323–341.
Moolgavkar, S.H., A. Dewanji, and D.J. Venson. 1988. A stochastic two-stage model for cancer risk assessment. I. The hazard function and the probability of tumor. Risk. Anal. 8:383–392.
Moolgavkar, S.H., E.G. Luebeck, M. de Gunst, R.E. Port, and M. Schwarz. 1990a. Quantitative analysis of enzyme-altered foci in rat hepatocarcinogenesis experiments—I: Single agent regimen. Carcinogenesis 11:1271–1278.
Moolgavkar, S.H., F.T. Cross, G. Luebeck, and G.E. Dagle. 1990b. A two-mutation model for radon-induced lung tumors in rats. Radiat. Res. 121:28–37.
Nathan, D.G. 1989. The beneficence of neonatal hematopoiesis. New Engl. J. Med. 321:1190–1191.
Norman, J.E., Jr. 1982. Breast cancer in women irradiated early in life. Pp. 433–450 in Banbury Report No. 11: Environmental Factors in Human Growth and Development, V.R. Hunt, M.K. Smith and D. Worth, eds. New York: Cold Spring Harbor Laboratory.
Owen, G.M., and J. Brozek. 1966. Influence of age, sex and nutrition on body composition during childhood and adolescence. Pp. 222–238 in Human Development, F. Falkner, ed. Philadelphia, Pa.: Saunders.
Rajewsky, M.P. 1985. Tumorigenesis by exogenous carcinogens: Role of target-cell proliferation and state of differentiation (development). Pp. 215–224 in Age-Related Factors in Carcinogenesis, Proceedings of Symposium, International Agency for Research on Cancer and N.N. Petrov Research Institute of Oncology, IARC Scientific Publications No. 58, A. Likhachev, V. Anisomov, and R. Montanaro, eds. Lyon, France: International Agency for Research on Cancer.
Rakic, P. 1970. Neuron-glia relationship during granule cell migration in developing cerebellar cortex. A Golgi and electromicroscopic study in macacus rhesus. J. Comp. Neurol. 141:283–312.
Rakic, P. 1972. Mode of cell migration to the superficial layers of fetal monkey neocortex. J. Comp. Neurol. 145:61–83.
Rodier, P.M. 1980. Chronology of neuron development: Animal studies and their clinical implications. Dev. Med. Child. Neurol. 22:525–545.
Singh, S.M., J.F. Toles, and J. Reaume. 1986. Genotype- and age-associated in vivo cytogenetic alterations following mutagenic exposures in mice. Can. J. Genet. Cytol. 28:286–293.
Swenberg, J.A., N. Fedtke, and L. Fishbein. 1992. Age-related differences in DNA adduct formation and carcinogenesis of vinyl chloride in rats. Pp. 163–171 in Similarities and Differences Between Children and Adults, P.S. Guzelian, C.J. Henry, and S.S. Olin, eds. Washington, D.C.: ILSI Press.
Tyan, M.L. 1982. Age-related increase in erythrocyte oxidant sensitivity. Mech. Ageing Dev. 20:25–32.
Vesell, E.S. 1982. Dynamically interacting genetic and environmental factors that affect the response of developing individuals to toxicants. Pp. 107–124 in Banbury Report No. 11: Environmental Factors in Human Growth and Development, V.R. Hunt, M.K. Smith, and D. Worth, eds. New York: Cold Spring Harbor Laboratory.
Weinstein, I.B. 1991. Mitogenesis is only one factor in carcinogenesis. Science 251:387–388.
Widdowson, E.M. 1968. Biological implications of body composition. Pp. 71–79 in Body Composition in Animals and Man, Proceedings of Symposium, Publication 1598. Washington, D.C.: National Academy of Sciences.
Widdowson, E.M., and J.W.T. Dickerson. 1960. The effect of growth and function on the chemical composition of soft tissues. Biochem. J. 77:30–43.
Widdowson, E.M., and J.W.T. Dickerson. 1964. Chemical composition of the body. Pp. 1–247 in Mineral Metabolism: An Advanced Treatise, C.L. Comar and F. Bronner, eds. New York: Academic Press.